Sustainable aviation fuels (SAFs) are pivotal in the fight against climate change and effectively reducing the aviation industry’s substantial carbon footprint. The aviation sector accounts for a significant portion of global greenhouse gas emissions, contributing around 2-3% of total CO2 emissions. With air travel demand on the rise, the imperative to cut these emissions has never been clearer.
SAFs are engineered to replace conventional fossil-based jet fuels, providing a powerful lower carbon alternative that can drastically lower lifecycle greenhouse gas emissions. By harnessing renewable feedstocks and leveraging advanced production technologies, SAFs can reduce CO2 emissions by up to 80% compared to traditional jet fuels. This shift is not just beneficial; it is essential for the aviation industry to meet international climate commitments, such as those established in the Paris Agreement, and to comply with initiatives like the Carbon Offsetting and Reduction Scheme for International Aviation (CORSIA), which pursues carbon-neutral growth in the sector.
What is Sustainable Aviation Fuel?
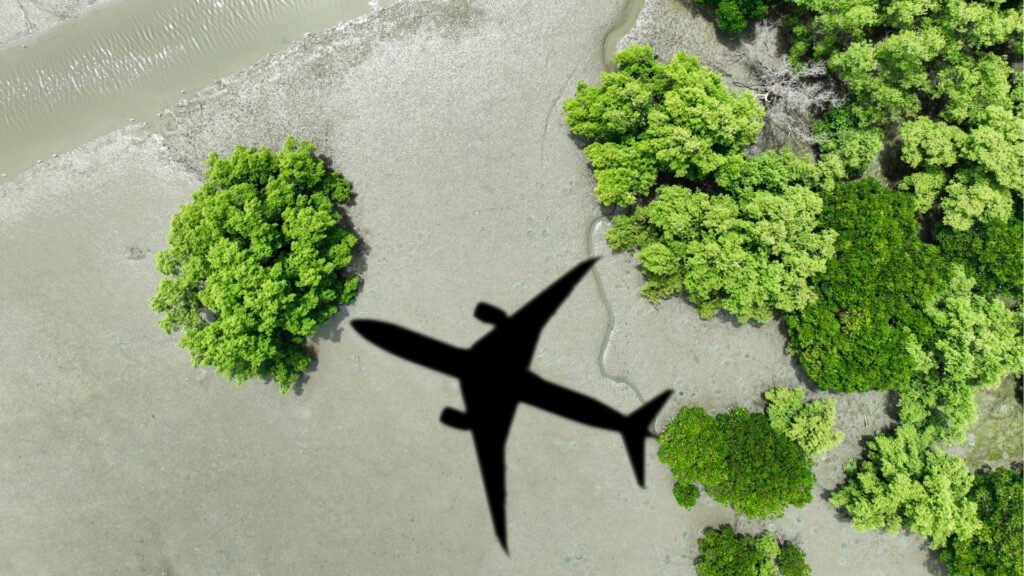
Sustainable Aviation Fuel (SAF) is a type of aviation fuel made from renewable or waste-derived sources. One of the key features of SAF is that it can be used as a “drop-in” fuel, which means it can be blended with traditional jet fuels without the need for modifications to existing aircraft engines or fuel infrastructure.
It encompasses a variety of fuel types, including:
- Biofuels: These fuels are derived from biological materials, including plant oils, agricultural residues, and used cooking oil.
- Synthetic Fuels: These are produced through chemical processes that convert renewable energy sources into liquid fuels. An example is Power-to-Liquid (PtL) fuels, which utilize green hydrogen and carbon dioxide (CO₂) captured from the atmosphere or industrial processes (Kurzawska-Pietrowicz & Jasiński, 2024).
The most promising technological pathways for producing sustainable aviation fuels.
1. Hydroprocessed Esters and Fatty Acids (HEFA):
- Efficiency: HEFA technology is currently the most mature and widely used, with a high technology readiness level (TRL) and fuel readiness level (FRL) of 9, indicating its readiness for commercial deployment.
- Cost: Production costs for HEFA are generally higher than conventional jet fuels, averaging at least 120% more, but it is considered economically viable due to its established infrastructure.
- Environmental Impact: HEFA can achieve significant reductions in greenhouse gas (GHG) emissions, with studies indicating reductions of at least 27% compared to fossil fuels (Watson et al., 2024).
2. Fischer-Tropsch (FT) Synthesis:
- Efficiency: FT synthesis converts biomass or other carbon sources into liquid hydrocarbons. It has shown promise in producing high-quality jet fuel.
- Cost: The costs associated with FT processes can be high due to the complexity of the technology and the need for significant capital investment in infrastructure.
- Environmental Impact: FT fuels can provide substantial GHG reductions, but the overall impact depends on the feedstock used and the energy sources for the conversion process (Ansell, 2023).
3. Alcohol-to-Jet (ATJ):
- Efficiency: ATJ processes convert alcohols (like ethanol) into jet fuel. This pathway is still under development but has potential for scalability.
- Cost: The cost of ATJ production is currently high, primarily due to the need for advanced processing technologies and the variability in feedstock prices.
- Environmental Impact: ATJ can reduce GHG emissions significantly, especially when using renewable feedstocks, but the overall sustainability depends on the lifecycle analysis of the feedstock (Kurzawska-Pietrowicz & Jasiński, 2024).
4. Power-to-Liquid (PtL):
- Efficiency: PtL technology utilizes renewable electricity to produce hydrogen, which is then combined with CO2 to create synthetic fuels. This pathway is promising for long-term sustainability.
- Cost: The costs are currently high due to the need for renewable energy sources and the infrastructure required for hydrogen production and storage.
- Environmental Impact: PtL has the potential for very low GHG emissions, especially when powered by renewable energy, but it requires significant advancements in technology and cost reduction to be viable at scale (Su-ungkavatin et al., 2023).
5. Biomass-to-Liquid (BTL):
- Efficiency: BTL processes convert biomass into liquid fuels through gasification and subsequent synthesis. This pathway can utilize a variety of feedstocks.
- Cost: The production costs can be high, influenced by feedstock availability and processing technologies.
- Environmental Impact: BTL can provide substantial GHG reductions, but the sustainability of the feedstock and the energy used in the process are critical factors (Emmanouilidou et al., 2023).
Comparison Summary:
- Efficiency: HEFA and FT processes currently lead in efficiency and readiness for commercial use, while ATJ and PtL are emerging technologies with potential for future scalability.
- Cost: All SAF production methods are generally more expensive than conventional jet fuels, with HEFA being the most economically viable at present. ATJ and PtL face higher costs due to technological and infrastructure challenges.
- Environmental Impact: All pathways have the potential to significantly reduce GHG emissions compared to fossil fuels, but the extent of these reductions varies based on feedstock, production methods, and energy sources used.
In conclusion, while HEFA stands out as the most promising pathway currently, ongoing research and development in ATJ, PtL, and BTL technologies are essential for achieving broader adoption and further reductions in the aviation sector’s carbon footprint (Dray et al., 2022), (Martinez-Valencia et al., 2021) & (Lau et al., 2024).
Types of feedstocks mostly suitable for SAF production.
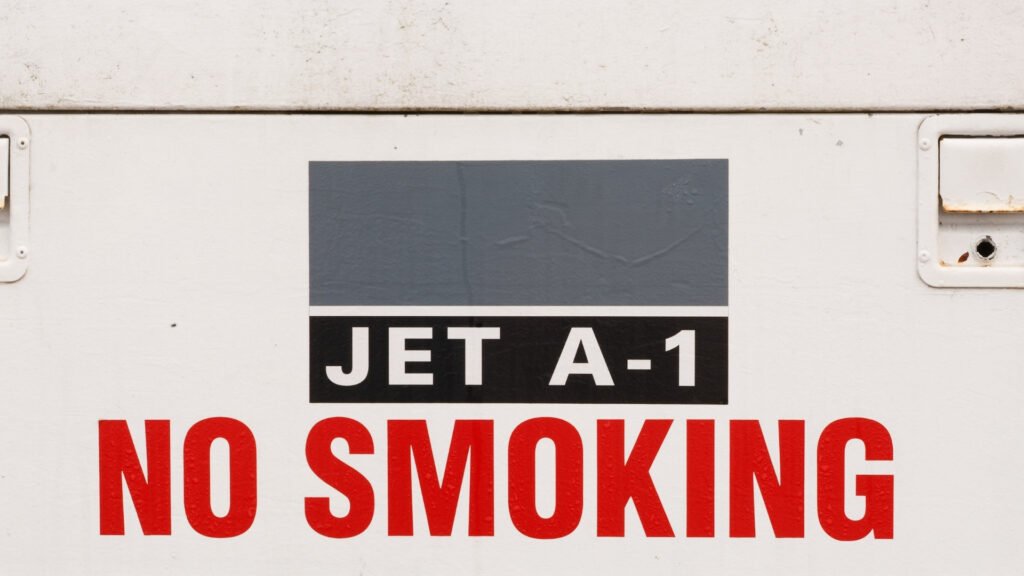
The production of Sustainable Aviation Fuels (SAFs) relies on various feedstocks, each with distinct characteristics, advantages, and challenges regarding availability and sustainability. The most suitable feedstocks for SAF production include:
Biomass Feedstocks:
- Non-Edible Oil Crops: Feedstocks such as camelina and jatropha are promising due to their high oil content and ability to grow in marginal lands. However, their cultivation can compete with food production and may lead to land-use changes that impact biodiversity (Afonso et al., 2023).
- Waste Oils: Used cooking oils and other waste fats are valuable feedstocks as they do not compete with food crops. However, their availability can be inconsistent and subject to market fluctuations (Doliente et al., 2020).
- Lignocellulosic Biomass: This includes agricultural residues, forestry residues, and dedicated energy crops. While they offer high potential yields and sustainability, the challenges lie in the complexity of processing and the need for advanced technologies to convert them into jet fuel (Lan et al., 2024).
Microalgae:
- Microalgae are considered a highly efficient feedstock due to their rapid growth rates and high lipid content. They can be cultivated on non-arable land and utilize wastewater, thus avoiding competition with food crops. However, the technology for large-scale cultivation and processing of microalgae is still in its infancy, presenting significant economic and technical challenges (Doliente et al., 2020).
Synthetic Feedstocks:
- Power-to-Liquid (PtL): This process utilizes renewable electricity to produce hydrogen, which is then combined with CO2 to create synthetic fuels. While this method has the potential for low lifecycle emissions, it requires significant infrastructure investment and the availability of sustainable CO2 sources (Zahid et al., 2024).
Challenges Associated with Availability and Sustainability.
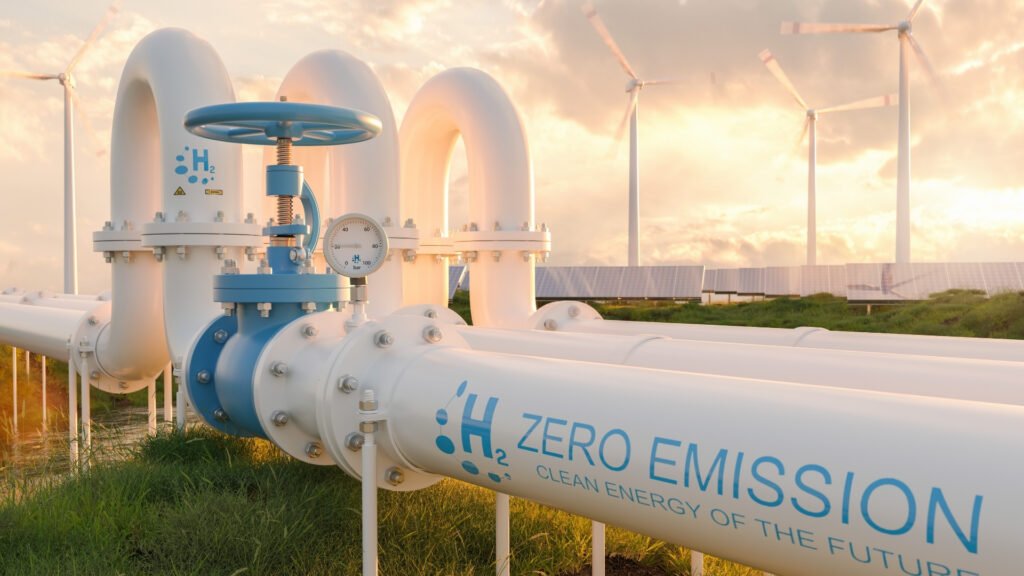
Feedstock Availability:
The supply of sustainable feedstocks can be limited by agricultural practices, climate conditions, and competition with food production. For instance, the cultivation of non-edible oil crops may lead to land-use changes that could negatively impact ecosystems (Afonso et al., 2023) & (Lan et al., 2024).
Economic Viability:
Many sustainable feedstocks, particularly advanced biofuels, are currently more expensive to produce than conventional fossil fuels. This price disparity can hinder the commercial viability of SAFs and necessitates government incentives or subsidies to encourage production (Watson et al., 2024).
Technological Maturity:
While some feedstocks and conversion technologies, such as Hydroprocessed Esters and Fatty Acids (HEFA), are more mature, others, like Fischer-Tropsch synthesis from lignocellulosic biomass, require further research and development to optimize efficiency and reduce costs (Zahid et al., 2024) & (Doliente et al., 2020).
Environmental Impact:
The sustainability of feedstocks must be assessed not only in terms of their carbon footprint but also regarding their impact on water resources, land use, and biodiversity. For example, the cultivation of certain crops for biofuels can lead to deforestation and habitat loss (Lau et al., 2024)
Economical implications of transitioning to SAFs
Production Costs
- Higher Costs Compared to Conventional Fuels: SAF production costs are generally higher than those of traditional fossil fuels. For instance, the minimum selling price (MSP) of SAF can range significantly depending on the production pathway and feedstock used, with estimates indicating costs from $1.8 to $9.9 per gallon gasoline equivalent (GGE) (Bhatt et al., 2023). Specific pathways like Hydroprocessed Esters and Fatty Acids (HEFA) have been noted to have production costs that can be two times that of fossil fuels (Qasem et al., 2024) & (Qasem et al., 2024).
- Feedstock Sensitivity: The costs are highly sensitive to feedstock prices, which can fluctuate based on availability and market demand. For example, the economic viability of SAF production from camelina oil showed a wide range of MSP depending on production conditions (Ahmad & Xu, 2021)
Market Dynamics
- Supply and Demand Imbalance: Despite the growing interest in SAF, production remains low, accounting for less than 1% of total jet fuel consumption as of recent years (Hopkins et al., 2023). The anticipated growth in aviation demand is expected to outpace the current SAF production capabilities, leading to a significant demand-supply gap.
- Investment in Infrastructure: The transition to SAF requires substantial investment in production facilities and infrastructure. For instance, to meet the European Union’s targets for SAF, an estimated 300 to 400 production plants would be necessary by 2030 (Becken et al., 2023). This highlights the need for coordinated efforts among stakeholders to develop the necessary infrastructure.
Financial Incentives and Subsidies
- Government Support: Financial incentives are crucial for making SAF economically viable. Policies such as tax exemptions, subsidies, and grants can help offset the higher production costs associated with SAF (Lau et al., 2024). For example, the U.S. Department of Transportation has proposed competitive grant programs to support SAF producers in establishing facilities (Bhatt et al., 2023).
- Market-Based Mechanisms: Initiatives like the Carbon Offsetting and Reduction Scheme for International Aviation (CORSIA) aim to create a market for SAF by providing financial mechanisms that can help airlines offset their carbon emissions through the use of SAF (Becken et al., 2023). This can enhance the attractiveness of SAF to airlines looking to meet regulatory requirements.
- Long-Term Contracts and Partnerships: Airlines are increasingly forming partnerships with SAF producers to secure long-term contracts, which can stabilize demand and encourage investment in SAF production (Hopkins et al., 2023). This approach can help mitigate the risks associated with fluctuating fuel prices and ensure a steady supply of SAF.
The current policies and regulations in place to support the development and adoption of SAFs.
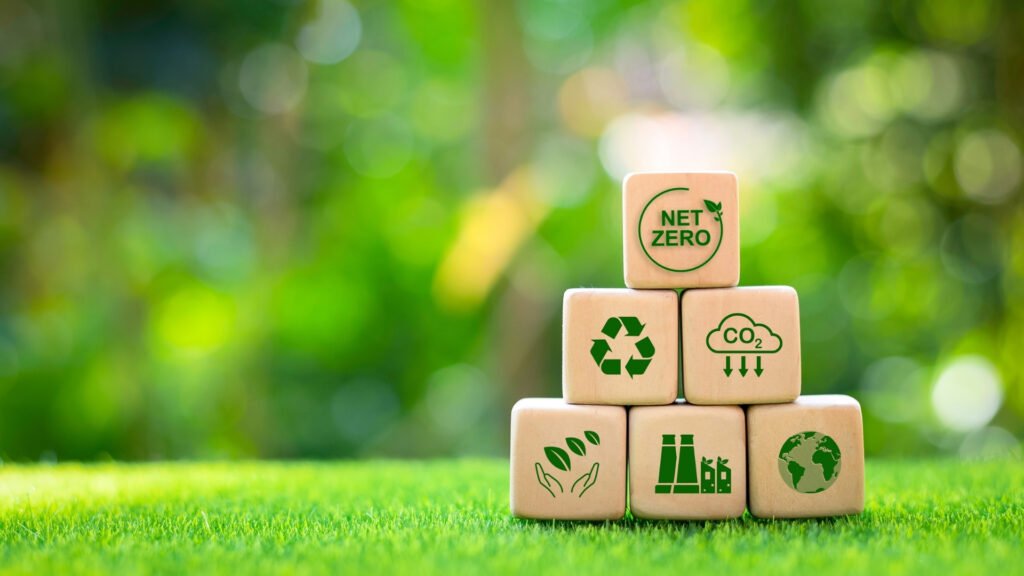
1. International Policies
- CORSIA (Carbon Offsetting and Reduction Scheme for International Aviation): Developed by the International Civil Aviation Organization (ICAO), CORSIA aims to stabilize international aviation emissions at 2020 levels by requiring airlines to offset emissions above this baseline. This scheme encourages the use of SAFs as a means to achieve compliance, thus promoting their adoption (Detsios et al., 2023).
- ICAO’s Global Framework for Aviation and Alternative Fuels (GFAAF): This framework provides guidelines for the development and deployment of SAFs, emphasizing the need for sustainability criteria and the reduction of lifecycle greenhouse gas emissions (Wang et al., 2024).
2. Regional and National Policies
- ReFuelEU Aviation Initiative: This European Union policy mandates that fuel suppliers ensure a minimum percentage of SAF in aviation fuel, starting at 2% in 2025 and increasing to 63% by 2050. This initiative is designed to stimulate the SAF market and ensure compliance with EU climate goals (Cabrera & de Sousa, 2022)& (Detsios et al., 2023).
- Sustainable Aviation Fuel User Group (SAFUG): Established in the U.S., this group promotes the development and use of SAFs through collaboration among stakeholders, including airlines, fuel producers, and government agencies. It aims to facilitate the transition to SAFs by sharing best practices and supporting research and development (Cui & Chen, 2024).
3. Financial Incentives and Support Mechanisms
- Tax Exemptions and Subsidies: Various countries, including the U.S., have implemented tax incentives to lower the cost of SAF production and encourage investment in SAF technologies. These financial mechanisms are crucial for making SAFs competitive with traditional fossil fuels (Cabrera & de Sousa, 2022)& (Cui & Chen, 2024)
- Grants and Funding Programs: Government grants, such as those provided by the U.S. Department of Energy, support research and development projects aimed at advancing SAF technologies and production methods (Shahriar & Khanal, 2022).
4. Effectiveness of Policies
- Market Growth: The implementation of these policies has led to an increase in SAF production and usage, although it still represents a small fraction of total aviation fuel consumption. For instance, SAF usage was less than 0.1% of total aviation fuel as of 2021, primarily due to high production costs and limited supply (Cui & Chen, 2024).
- Challenges in Implementation: Despite the supportive policies, challenges remain, including the maturity of production pathways, the high cost of SAFs (which can be 2-8 times that of conventional fuels), and the need for further technological advancements and economies of scale to make SAFs more accessible (Cui & Chen, 2024), (Whittle et al., 2024).
The current state of technological readiness for various SAF production methods.
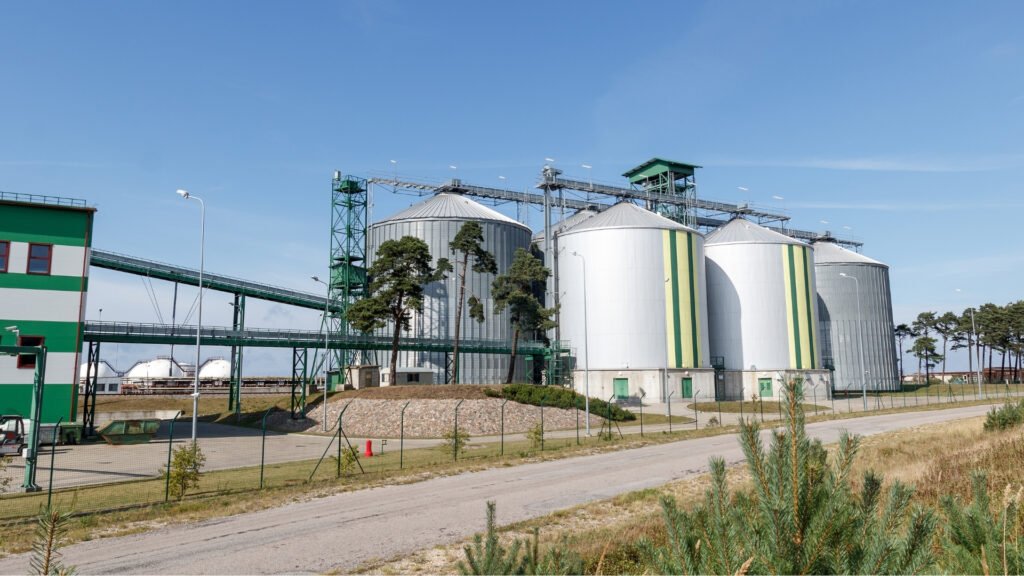
1. Technological Readiness Levels (TRL)
- Hydroprocessed Esters and Fatty Acids (HEFA): This pathway is the most commercially mature and widely used for SAF production. It has been certified under ASTM D7566 and is capable of producing fuels that can be blended with conventional jet fuel up to 50% without requiring modifications to existing aircraft engines (Petersen et al., 2021). HEFA technology is currently in commercial operation, with several production facilities already established.
- Fischer-Tropsch (FT) Synthesis: This method is also recognized but is less mature than HEFA. It involves converting syngas (derived from biomass or waste) into liquid hydrocarbons. While some pilot projects exist, the technology requires further development to enhance efficiency and reduce costs (Qasem et al., 2024) & (Zahid et al., 2024).
- Alcohol-to-Jet (ATJ): This pathway is in the demonstration phase, with several projects underway to validate its commercial viability. ATJ processes convert alcohols (like ethanol) into jet fuel, but they face challenges related to production costs and scalability (Martinez-Valencia et al., 2021)
- Direct Sugar to Hydrocarbon (DSHC): This method is still in the early stages of development and has not yet reached commercial readiness. It involves converting sugars directly into hydrocarbons suitable for aviation fuel (Martinez-Valencia et al., 2021)
- Catalytic Hydrothermolysis (CH): This is a newer technology that is still in the prototype stage. It aims to convert biomass into jet fuel but requires further research to optimize the process and improve yields. (Martinez-Valencia et al., 2021)
2. Advancements Needed for Widespread Adoption
- Cost Reduction: The production costs of SAF remain significantly higher than those of conventional jet fuels, often 2 to 8 times more expensive (Cui & Chen, 2024) , (Chen et al., 2024). Advancements in technology, economies of scale, and improved feedstock availability are essential to make SAF economically competitive.
- Feedstock Availability: Ensuring a reliable and sustainable supply of feedstocks is critical. This includes developing pathways that utilize waste materials and non-food crops to minimize competition with food production (Qasem et al., 2024) & (Zahid et al., 2024).
- Infrastructure Development: The existing infrastructure for fuel distribution and storage at airports needs to be adapted to accommodate SAF. This includes retrofitting facilities to handle different fuel properties and ensuring compatibility with current aviation systems (Chen et al., 2024) & (Petersen et al., 2021).
- Regulatory Support and Incentives: Stronger government policies and incentives are necessary to encourage investment in SAF technologies. This includes establishing blending mandates, carbon pricing mechanisms, and subsidies to lower the financial barriers for SAF adoption (Detsios et al., 2023)& (Dray et al., 2022)
- Research and Development: Continued R&D is crucial to improve the efficiency of production processes, enhance the quality of SAF, and develop new pathways that can offer better economic and environmental performance (Qasem et al., 2024),(Detsios et al., 2023).
How do airlines and consumers perceive SAFs, and what factors influence their acceptance and adoption in the aviation market?
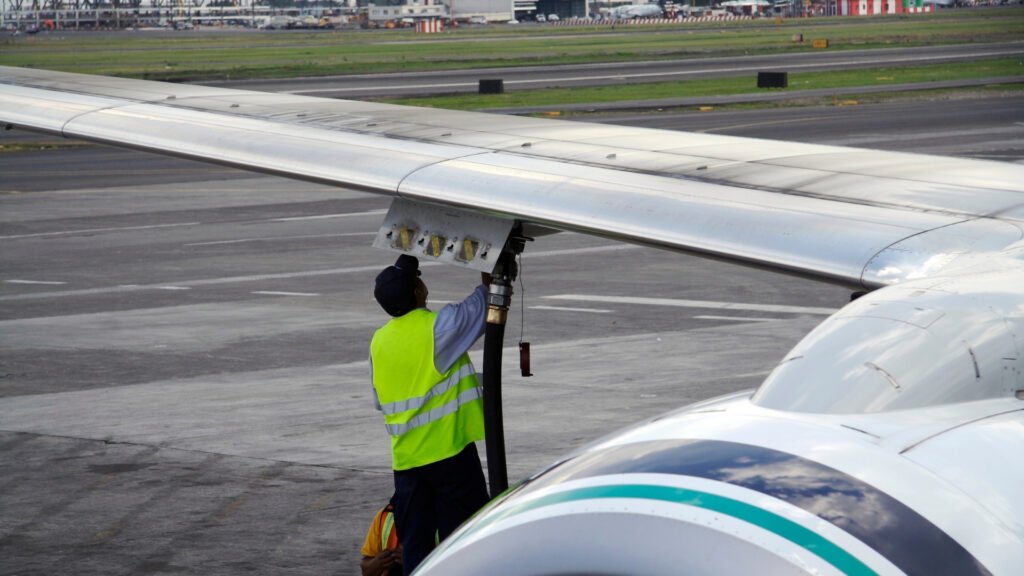
1. Airlines’ Perception of SAFs
- Cost Concerns: Airlines are primarily concerned about the high production costs of SAFs, which can be 2 to 8 times higher than conventional jet fuels (Cui & Chen, 2024). This cost disparity makes it challenging for airlines to adopt SAFs without significant financial incentives or government support.
- Regulatory Compliance: Airlines recognize the necessity of SAFs in meeting regulatory requirements and sustainability goals. The International Air Transport Association (IATA) has set ambitious targets for reducing carbon emissions, and SAFs are seen as a critical component in achieving these targets (Detsios et al., 2023).
- Brand Image and Corporate Responsibility: Many airlines are motivated to adopt SAFs to enhance their brand image and demonstrate corporate responsibility. For instance, airlines like Cathay Pacific and Alaska Airlines promote their use of SAFs as part of their commitment to sustainability, appealing to environmentally conscious consumers (Hopkins et al., 2023).
2. Consumers’ Perception of SAFs
- Environmental Awareness: Consumers are increasingly aware of the environmental impact of air travel and are generally supportive of initiatives that reduce carbon emissions. Studies indicate that passengers are willing to pay a premium for flights that utilize SAFs, especially if they perceive a direct environmental benefit (Chen et al., 2024).
- Safety and Reliability Concerns: While consumers are supportive of SAFs, there are concerns regarding the safety and reliability of these fuels. The need for rigorous certification processes and proven performance in existing aircraft is crucial for consumer acceptance (Kramer et al., 2022).
3. Factors Influencing Acceptance and Adoption
- Economic Incentives: Government policies and financial incentives play a significant role in promoting the adoption of SAFs. Tax exemptions, subsidies, and mandates for SAF usage can help offset the higher costs associated with these fuels (Shahriar & Khanal, 2022) & (Cabrera & de Sousa, 2022).
- Public Relations and Marketing: Airlines that effectively communicate the benefits of SAFs and their commitment to sustainability can enhance consumer acceptance. Public relations campaigns that highlight the environmental benefits of SAFs can positively influence consumer perceptions (Watson et al., 2024).
- Technological Readiness and Infrastructure: The availability of infrastructure to support SAF production and distribution is critical. Airlines are more likely to adopt SAFs if there is a robust supply chain and production facilities in place (Shahriar & Khanal, 2022).
- Stakeholder Engagement: Engaging various stakeholders, including governments, fuel producers, and consumers, is essential for building a supportive ecosystem for SAF adoption. Collaborative efforts can help address concerns and promote the benefits of SAFs (Okolie et al., 2023)
Potential environmental benefits and drawbacks of using SAFs.
Environmental Benefits of SAFs
- Reduction in Greenhouse Gas Emissions: SAFs can significantly reduce lifecycle greenhouse gas emissions compared to conventional fossil fuels. Studies indicate that SAFs can achieve reductions of up to 80% in lifecycle GHG emissions, contributing to climate change mitigation efforts (Wang et al., 2024) & (Ansell, 2023).
- Utilization of Waste Feedstocks: Many SAF production pathways utilize waste materials, such as municipal solid waste, agricultural residues, and used cooking oils. This approach not only helps in waste management but also reduces the need for virgin feedstocks, thereby minimizing land use changes and preserving natural habitats (Okolie et al., 2023), (Yilmaz & Atmanli, 2017).
- Biodiversity Preservation: By using non-food feedstocks and waste materials, SAF production can potentially avoid the negative impacts associated with food crop cultivation, such as habitat destruction and biodiversity loss. This is particularly relevant when compared to first-generation biofuels that compete with food crops for land (Ansell, 2023), (Okolie et al., 2023).
- Water Resource Management: SAFs derived from waste or non-food crops can reduce the pressure on freshwater resources. For instance, certain feedstocks like halophytes can be cultivated in saline conditions, thus not competing with freshwater resources needed for food production (Afonso et al., 2023).
Environmental Drawbacks of SAFs
- Land Use Change: The cultivation of biomass for SAF can lead to land use changes, which may result in habitat destruction and loss of biodiversity if not managed sustainably. The conversion of forests or grasslands to biomass production areas can have significant ecological impacts (Ansell, 2023), (Yilmaz & Atmanli, 2017).
- Competition for Resources: While SAFs can utilize waste materials, there is still a risk that the demand for biomass could lead to competition for land and water resources, particularly if food crops are displaced. This could exacerbate food security issues and lead to increased pressure on ecosystems (Ansell, 2023), (Okolie et al., 2023).
- Water Consumption: The production of certain biomass feedstocks can be water-intensive, potentially leading to depletion of local water resources. This is particularly concerning in arid regions where water scarcity is already a significant issue (Ansell, 2023), (Yilmaz & Atmanli, 2017).
- Indirect Land Use Change (ILUC): The production of SAFs can lead to indirect land use changes, where the cultivation of biofuel crops in one area leads to the displacement of food crops to another area, potentially resulting in deforestation and habitat loss elsewhere (Ansell, 2023), (Yilmaz & Atmanli, 2017).
How can collaboration between stakeholders, including governments, industry, and academia, be fostered to accelerate the development and deployment of SAFs?
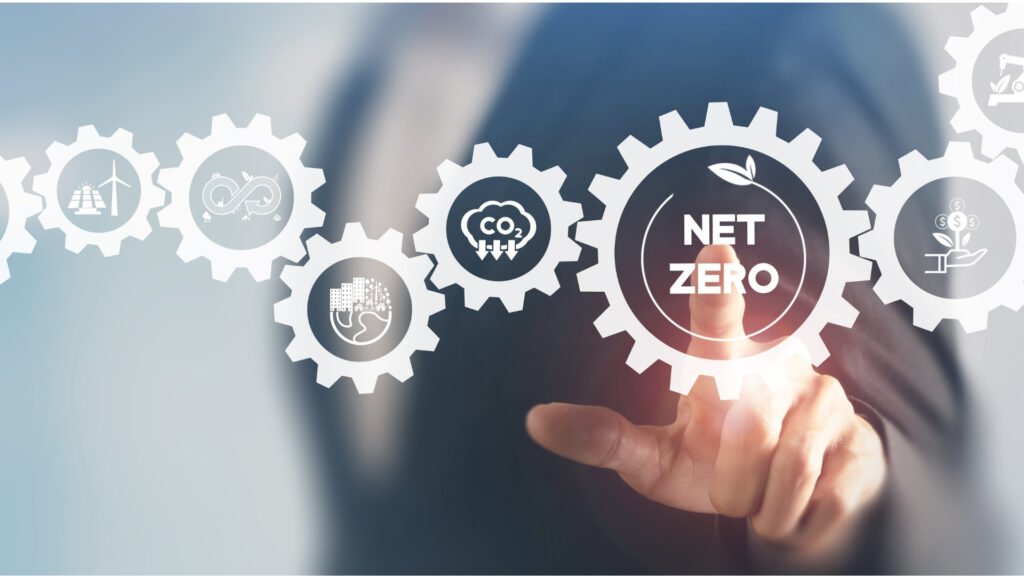
Collaboration between stakeholders, including governments, industry, and academia, is essential for accelerating the development and deployment of Sustainable Aviation Fuels (SAFs). Here are several strategies to foster such collaboration:
1. Establishing Public-Private Partnerships
- Joint Research Initiatives: Governments can partner with industry and academic institutions to fund and conduct research on SAF technologies. This can include joint projects that leverage the expertise of each sector to address technical challenges and improve production processes (Chai & Zhou, 2022).
- Funding and Incentives: Governments can provide financial incentives, such as grants or tax credits, to encourage private sector investment in SAF research and development. This can help mitigate the high costs associated with SAF production (Watson et al., 2024).
2. Creating Collaborative Frameworks
- Industry Consortia: Forming consortia that include airlines, fuel producers, and technology developers can facilitate knowledge sharing and resource pooling. These groups can work together to establish common goals, share best practices, and develop standardized protocols for SAF production and use (Emmanouilidou et al., 2023).
- Regulatory Collaboration: Engaging stakeholders in the regulatory process can ensure that policies are conducive to SAF development. This includes involving industry representatives in discussions about fuel standards and sustainability criteria (Gan et al., 2023).
3. Enhancing Knowledge Transfer
- Workshops and Conferences: Organizing events that bring together stakeholders from different sectors can foster dialogue and collaboration. These events can focus on sharing research findings, discussing challenges, and exploring innovative solutions for SAF development (Karakoc et al., 2024).
- Educational Programs: Academic institutions can develop training programs and workshops aimed at educating industry professionals about SAF technologies and sustainability practices. This can help build a knowledgeable workforce that is equipped to advance SAF initiatives (Sharno & Hiloidhari, 2024).
4. Leveraging Technology and Innovation
- Innovation Hubs: Establishing innovation hubs or incubators that focus on SAF technologies can provide a collaborative space for startups, researchers, and established companies to work together on developing new solutions (Su-ungkavatin et al., 2023).
- Digital Platforms: Utilizing digital platforms for collaboration can enhance communication and project management among stakeholders. These platforms can facilitate data sharing, project tracking, and collaborative research efforts (Mueller et al., 2024)
5. Aligning Goals and Objectives
- Shared Vision: Developing a shared vision for the future of aviation that includes SAFs can help align the interests of various stakeholders. This can involve setting common targets for emissions reductions and sustainability that all parties can work towards (Rogachuk & Okolie, 2024).
- Policy Alignment: Ensuring that government policies support the goals of the aviation industry and academia regarding SAF development can create a more favorable environment for collaboration. This includes aligning regulations with industry needs and sustainability objectives (Watson et al., 2024).
References.
Afonso, F., Sohst, M., Diogo, C. M. A., Rodrigues, S. S., Ferreira, A., Ribeiro, I., Marques, R., Rego, F. F. C., Sohouli, A., Portugal-Pereira, J., Policarpo, H., Soares, B., Ferreira, B., Fernandes, E. C., Lau, F., & Suleman, A. (2023). Strategies towards a more sustainable aviation: A systematic review. Progress in aerospace sciences, 137, 100878. https://doi.org/10.1016/j.paerosci.2022.100878
Ahmad, S., & Xu, B. (2021). A cognitive mapping approach to analyse stakeholders’ perspectives on sustainable aviation fuels. Transportation research. Part D, Transport and environment, 100, 103076. https://doi.org/10.1016/j.trd.2021.103076
Ansell, P. J. (2023). Review of sustainable energy carriers for aviation: Benefits, challenges, and future viability. Progress in aerospace sciences, 141, 100919. https://doi.org/10.1016/j.paerosci.2023.100919
Becken, S., Mackey, B., & Lee, D. S. (2023). Implications of preferential access to land and clean energy for Sustainable Aviation Fuels. The Science of the total environment, 886, 163883-163883. https://doi.org/10.1016/j.scitotenv.2023.163883
Bhatt, A. H., Zhang, Y., Milbrandt, A., Newes, E., Moriarty, K., Klein, B., & Tao, L. (2023). Evaluation of performance variables to accelerate the deployment of sustainable aviation fuels at a regional scale. Energy conversion and management, 275, 116441. https://doi.org/10.1016/j.enconman.2022.116441
Cabrera, E., & de Sousa, J. M. M. (2022). Use of Sustainable Fuels in Aviation—A Review. Energies (Basel), 15(7), 2440. https://doi.org/10.3390/en15072440
Chai, N., & Zhou, W. (2022). A novel hybrid MCDM approach for selecting sustainable alternative aviation fuels in supply chain management. Fuel (Guildford), 327, 125180. https://doi.org/10.1016/j.fuel.2022.125180
Chen, R., Yang, H., Wang, K., & Jiang, C. (2024). Impacts of a sustainable aviation fuel mandate on airline competition — Full-service carrier vs. low-cost carrier. Transportation research. Part B: methodological, 190, 103098. https://doi.org/10.1016/j.trb.2024.103098
Cui, Q., & Chen, B. (2024). Cost-benefit analysis of using sustainable aviation fuels in South America. Journal of Cleaner Production, 435, 140556. https://doi.org/10.1016/j.jclepro.2024.140556
Detsios, N., Theodoraki, S., Maragoudaki, L., Atsonios, K., Grammelis, P., & Orfanoudakis, N. G. (2023). Recent Advances on Alternative Aviation Fuels/Pathways: A Critical Review. Energies (Basel), 16(4), 1904. https://doi.org/10.3390/en16041904
Doliente, S. S., Narayan, A., Tapia, J. F. D., Samsatli, N. J., Zhao, Y., & Samsatli, S. (2020). Bio-aviation Fuel: A Comprehensive Review and Analysis of the Supply Chain Components. Frontiers in energy research, 8. https://doi.org/10.3389/fenrg.2020.00110
Dray, L., Schäfer, A. W., Grobler, C., Falter, C., Allroggen, F., Stettler, M. E. J., & Barrett, S. R. H. (2022). Cost and emissions pathways towards net-zero climate impacts in aviation. Nature climate change, 12(10), 956-962. https://doi.org/10.1038/s41558-022-01485-4
Emmanouilidou, E., Mitkidou, S., Agapiou, A., & Kokkinos, N. C. (2023). Solid waste biomass as a potential feedstock for producing sustainable aviation fuel: A systematic review. Renewable energy, 206, 897-907. https://doi.org/10.1016/j.renene.2023.02.113
Gan, C., Ma, Q., Bao, S., Wang, X., Qiu, T., & Ding, S. (2023). Discussion of the Standards System for Sustainable Aviation Fuels: An Aero-Engine Safety Perspective. Sustainability, 15(24), 16905. https://doi.org/10.3390/su152416905
Hopkins, D., Gössling, S., Cohen, S., Hanna, P., & Higham, J. E. S. (2023). Aeromasculinities and the fallacy of sustainable aviation. Energy research & social science, 106, 103319. https://doi.org/10.1016/j.erss.2023.103319
Karakoc, T. H., Das, R., Ekmekci, I., Dalkiran, A., & Ercan, A. H. (2024). Green Approaches in Sustainable Aviation : Proceedings of International Symposium on Sustainable Aviation 2022 (1st 2024. ed.). Springer Nature Switzerland. https://doi.org/10.1007/978-3-031-33118-3
Kramer, S., Andac, G., Heyne, J., Ellsworth, J., Herzig, P., & Lewis, K. C. (2022). Perspectives on Fully Synthesized Sustainable Aviation Fuels: Direction and Opportunities. Frontiers in energy research, 9. https://doi.org/10.3389/fenrg.2021.782823
Kurzawska-Pietrowicz, P., & Jasiński, R. (2024). A Review of Alternative Aviation Fuels. Energies (Basel), 17(16), 3890. https://doi.org/10.3390/en17163890
Lan, K., Cruz, D., Li, J., Agyei Boakye, A. A., Park, H., Tiller, P., Mittal, A., Johnson, D. K., Park, S., & Yao, Y. (2024). Life-Cycle Assessment of Sustainable Aviation Fuel Derived from Paper Sludge. ACS sustainable chemistry & engineering, 12(22), 8379-8390. https://doi.org/10.1021/acssuschemeng.4c00795
Lau, J. I. C., Wang, Y. S., Ang, T., Seo, J. C. F., Khadaroo, S. N. B. A., Chew, J. J., Ng Kay Lup, A., & Sunarso, J. (2024). Emerging technologies, policies and challenges toward implementing sustainable aviation fuel (SAF). Biomass & bioenergy, 186, 107277. https://doi.org/10.1016/j.biombioe.2024.107277
Martinez-Valencia, L., Garcia-Perez, M., & Wolcott, M. P. (2021). Supply chain configuration of sustainable aviation fuel: Review, challenges, and pathways for including environmental and social benefits. Renewable & sustainable energy reviews, 152, 111680. https://doi.org/10.1016/j.rser.2021.111680
Mueller, T., Winter, E., & Grote, U. (2024). Economic impacts of power-to-liquid fuels in aviation: A general equilibrium analysis of production and utilization in Germany. Energy conversion and management. X, 23, 100632. https://doi.org/10.1016/j.ecmx.2024.100632
Okolie, J. A., Awotoye, D., Tabat, M. E., Okoye, P. U., Epelle, E. I., Ogbaga, C. C., Güleç, F., & Oboirien, B. (2023). Multi-criteria decision analysis for the evaluation and screening of sustainable aviation fuel production pathways. iScience, 26(6), 106944-106944. https://doi.org/10.1016/j.isci.2023.106944
Petersen, A. M., Chireshe, F., Okoro, O., Gorgens, J., & Van Dyk, J. (2021). Evaluating refinery configurations for deriving sustainable aviation fuel from ethanol or syncrude. Fuel processing technology, 219, 106879. https://doi.org/10.1016/j.fuproc.2021.106879
Qasem, N. A. A., Mourad, A., Abderrahmane, A., Said, Z., Younis, O., Guedri, K., & Kolsi, L. (2024). A recent review of aviation fuels and sustainable aviation fuels. Journal of thermal analysis and calorimetry, 149(10), 4287-4312. https://doi.org/10.1007/s10973-024-13027-5
Rogachuk, B. E., & Okolie, J. A. (2024). Comparative assessment of pyrolysis and Gasification-Fischer Tropsch for sustainable aviation fuel production from waste tires. Energy conversion and management, 302, 118110. https://doi.org/10.1016/j.enconman.2024.118110
Shahriar, M. F., & Khanal, A. (2022). The current techno-economic, environmental, policy status and perspectives of sustainable aviation fuel (SAF). Fuel (Guildford), 325, 124905. https://doi.org/10.1016/j.fuel.2022.124905
Sharno, M. A., & Hiloidhari, M. (2024). Social sustainability of biojet fuel for net zero aviation. Energy for sustainable development, 79, 101419. https://doi.org/10.1016/j.esd.2024.101419
Su-ungkavatin, P., Tiruta-Barna, L., & Hamelin, L. (2023). Biofuels, electrofuels, electric or hydrogen?: A review of current and emerging sustainable aviation systems. Progress in energy and combustion science, 96, 101073. https://doi.org/10.1016/j.pecs.2023.101073
Wang, B., Ting, Z. J., & Zhao, M. (2024). Sustainable aviation fuels: Key opportunities and challenges in lowering carbon emissions for aviation industry. Carbon Capture Science & Technology, 13, 100263. https://doi.org/10.1016/j.ccst.2024.100263
Watson, M. J., Machado, P. G., da Silva, A. V., Saltar, Y., Ribeiro, C. O., Nascimento, C. A. O., & Dowling, A. W. (2024). Sustainable aviation fuel technologies, costs, emissions, policies, and markets: A critical review. Journal of Cleaner Production, 449, 141472. https://doi.org/10.1016/j.jclepro.2024.141472
Whittle, J. W., Callander, K., Akure, M., Kachwala, F., & Koh, S. C. L. (2024). A new high-level life cycle assessment framework for evaluating environmental performance: An aviation case study. Journal of Cleaner Production, 471, 143440. https://doi.org/10.1016/j.jclepro.2024.143440
Yilmaz, N., & Atmanli, A. (2017). Sustainable alternative fuels in aviation. Energy (Oxford), 140, 1378-1386. https://doi.org/10.1016/j.energy.2017.07.077
Zahid, I., Nazir, M. H., Chiang, K., Christo, F., & Ameen, M. (2024). Current outlook on sustainable feedstocks and processes for sustainable aviation fuel production. Current opinion in green and sustainable chemistry, 49, 100959. https://doi.org/10.1016/j.cogsc.2024.100959